The Radius Gap
In 2017 , the California Kepler Survey ( Fulton et al 2017) revealed that the radii of small planets has a bimodal distribution (see below). One explanation is the photoevaporation by high energy radiation. EUV and X-ray can deposit heat into the atmosphere and drives an outflow that leads to mass loss ( Owen and Wu 2013, Lopez and Fortney 2014, Owen and Wu 2017). The lower peak of the radius distribtion comprises of rocky cores that have their H/He envelope completely stripped off by photoevaporation. Since high energy radiation diminishes after the first 100 Myr of the star's lifetime ( Ribas et al 2005), larger planets with thicker H/He envelope may still keep a few % envelope by the time high energy radiation shuts down. The H/He envelope of a few % envelope is able to significantly inflate these planets' radii pushing them to the higher peak of the radius distribution.
An alternative explanation was proposed by Ginzburg et al 2017: the luminosity of the cooling, contracting core alone is able to drive significant atmospheric loss sculpting out the observed radius gap. Ginzburg et al 2017 further proposed that a simple way to distinguish 1) photoevaporation and 2) core-powered atmospheric loss is to examine the radius distribution across different stellar types. Photoevaporation, which is powered by the high-energy radiation, should see a strong dependence on stellar types, given that different types of stars give off different proportions of their energy in high-energy radiation. On the other hand, core-powered atmospheric erosion should be the same as long as the bolometric insolation (i.e. planets' equilibrium temperature) is held constant.

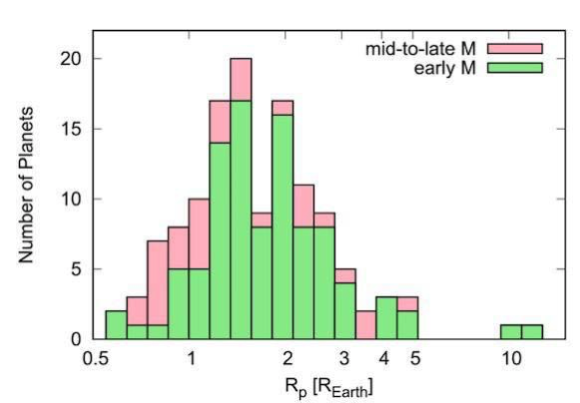
To compare photoevaporation and core-powered mass loss, we looked at the radius distribution of planets around M dwarfs which are known to be more active than Sun-like stars and give off a larger fraction of their energy in high energy radiation. In our recent work led by my collaborator Teruyuki Hirano (Hirano, Dai et al 2017 ) we reported 16 new planets around M dwarfs which represents a substantial addition to existing sample ~80 planets around M dwarf. Since spectroscopic parameters of M dwarf are notoriously difficult to obtain. We also performed a uniform analysis of all known M dwarf planets. The radius gap is also present in M dwarf planets indicating that whatever process responsible for the radius gap must also operate for M dwarf planets.
Photoevaporation Desert
We then investigated the location of the so-called “photoevaporation desert” which is a lack of planet between 2-4R⊕ at the highest insolation level. For Sun-ike the photoevaporation desert identified by Lundkvist et al 2016 has a boundary at about 650 Earth insolation level F⊕.
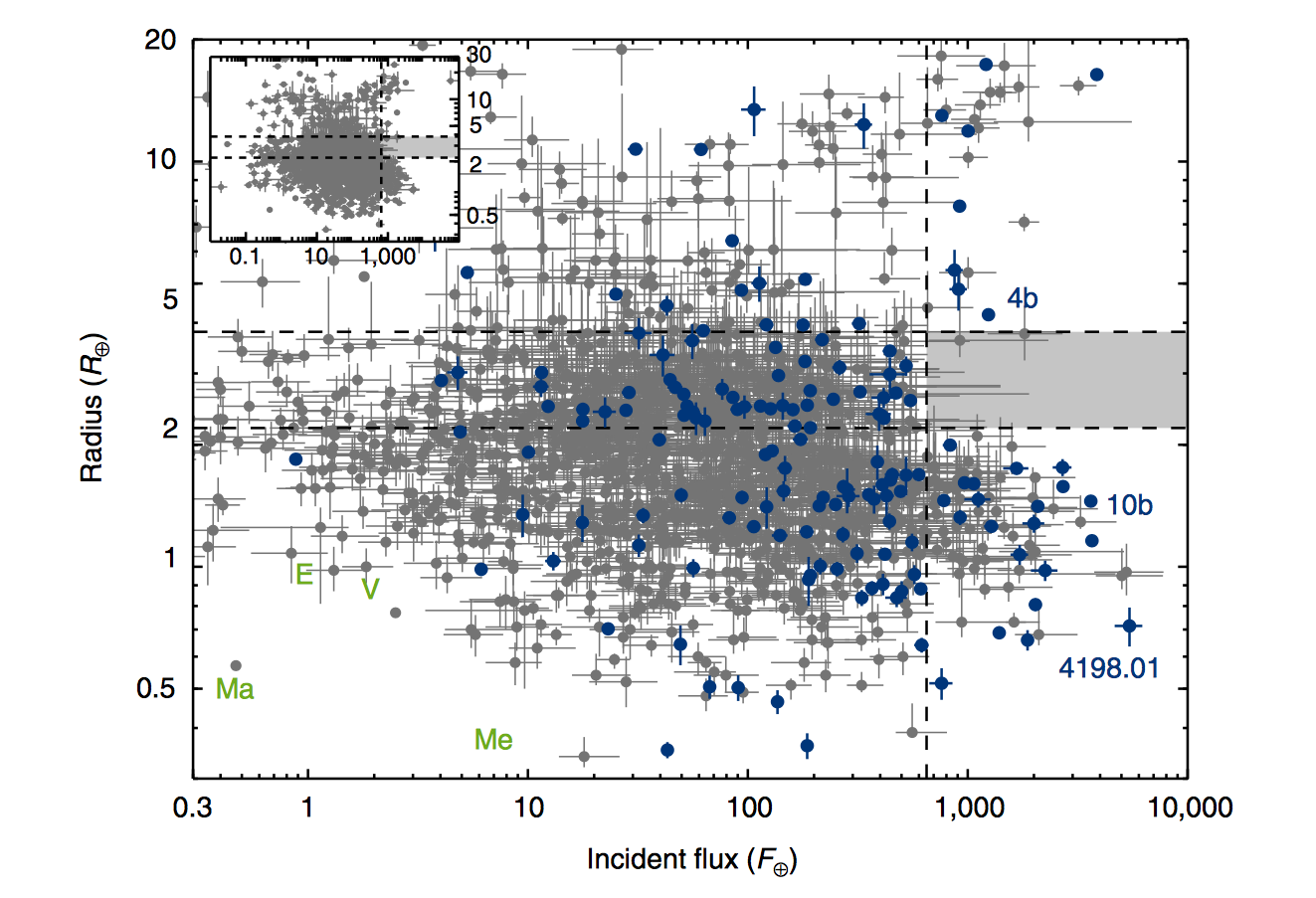
We made similar plots for M-dwarf planets splitting between early M dwarf and mid-late M dwarfs (Teff = 3500K). Note that the blue box corresponds to the “photoevaporation desert” identified by Lundkvist et al 2016 around Sun-like stars. We can see that the “photoevaporation desert” occurs at a lower bolometric insolation level compared to Sun-like stars. In other words, M dwarfs seem to be more effective at reducing the size of their planets, a telltale sign that photoevaporation is the main driver for the radius gap. In the opposing case of core-powered mass loss, the photoevaporation desert should occur at the same bolometric insolation level. This should not be a selection effect because closer-in but larger planets should be much easier to detect from transit surveys. Also, notice the three outliers: HATS-6b and Kepler-45b are hot Jupiter where gravity is strong enough that photoevaporation can not operate efficiently. K2-33 is a young system ~10 Myr; it is likely still undergoing photoevaporation.
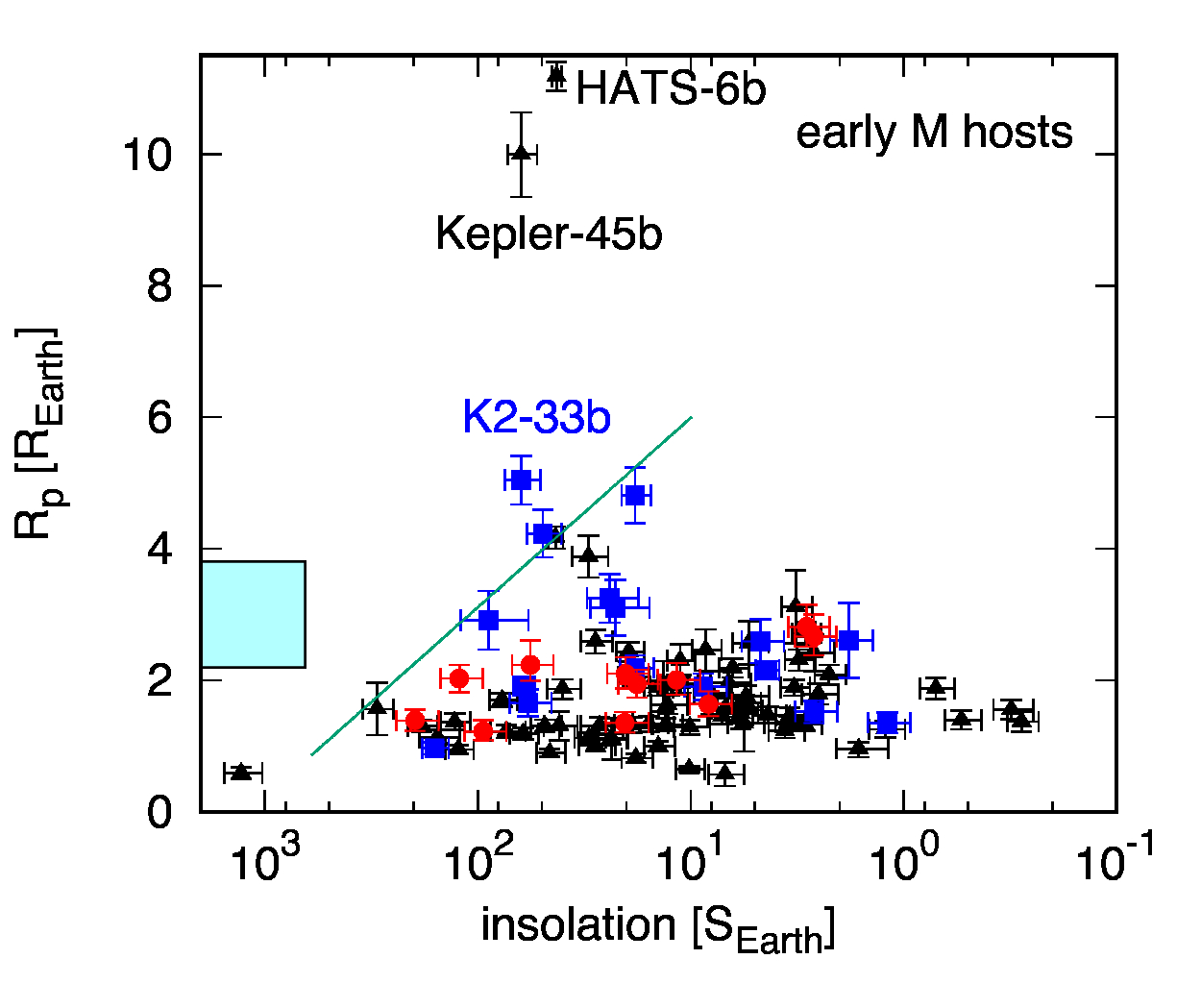
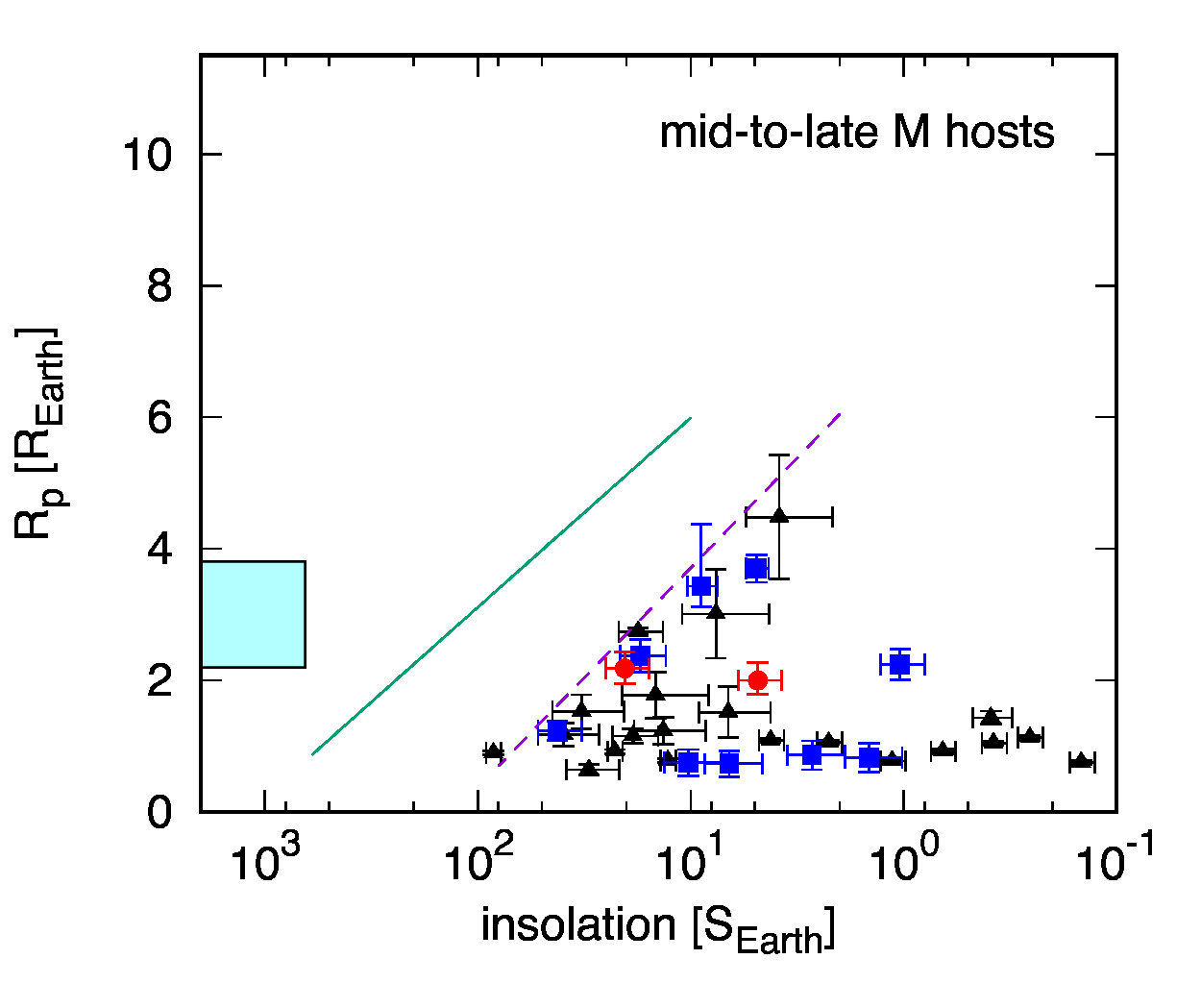